Attosecond physics: observing electrons with a magnifying glass
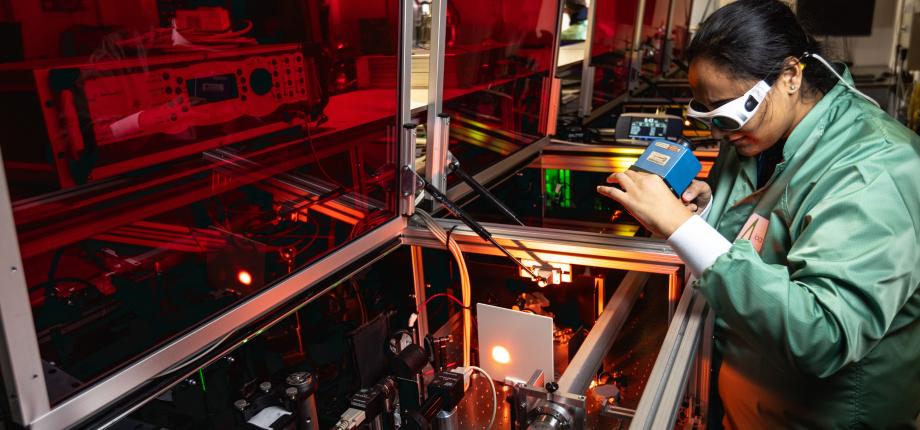
In 2001, the journal Science published an article showing, for the first time, how to measure and characterize trains of light pulses of minute duration. Pierre Agostini, then a researcher at CEA Saclay and co-winner of the 2023 Nobel Prize in Physics, and his team conducted the experiment at LOA. "In the 1990s and early 2000s, the LOA was one of the few facilities in the world to have an intense laser with a high firing rate for measuring these pulses", explains Stéphane Sebban, the laboratory's director. This was a crucial step in the ongoing history of attosecond physics.
On the electron scale
Taking an interest in attosecond physics is to dive into the heart of fundamental physics, that which explores atoms, electrons, photons... "In the hydrogen atom, the electron orbits the nucleus in an ultra-short time, 150 attoseconds", explains LOA researcher Rodrigo Lopez-Martens. But an attosecond is 10-18s. A billionth of a billionth of a second. There are, for example, a billion billion attoseconds in the time it takes to pronounce the word attosecond. Vertiginous. "If we want to understand the dynamics of the electron, we need "cameras" with "shutter speeds" high enough to capture the motion of this particle".
A quick reminder of physics. Light is made up of waves - vibrations of electric and magnetic fields. These vibrations are characterized, among other things, by different wavelengths or frequencies (characteristics that correspond to the different colors of visible light). The shorter the wavelength, the higher the frequency and the faster the light wave vibrates. So, to produce a short pulse, you'd think you'd only need to generate a single "cycle" of the wave. Lasers emitting light at visible wavelengths can generate pulses lasting less than a few femtoseconds (10-15s). This is short enough to observe the dynamics of atoms and molecules, but not electrons. "For even shorter flashes, we need very short wavelengths. This is equivalent to a laser emitting outside the visible spectrum, in the far ultraviolet, the XUV. But this didn't exist at the time of the first experiments, and is still very rare today. So we had to find another strategy", explains Stefan Haessler.
35 years of attosecond physics
Anne L'Huillier discovered this strategy by chance in 1988, while working as a researcher at the CEA. By aiming an infrared laser at a noble gas as part of another experiment, the now co-Nobel Prize winner for physics observed the production of high-order harmonics, i.e. the production of light whose frequencies are very high multiples of the initial laser frequency (article 1). Scientists soon realized that this phenomenon could be used to create short pulses. For Rodrigo Lopez-Martens, "it's a bit like banging on a drum. If we analyze the resulting sound spectrum, we see that there are in fact a multitude of waves of different frequencies. And when they come into phase, we hear a short sound". Here, the gas and its electrons are the drumhead and the laser the drumstick. As the laser passes through the gas, it produces a multitude of waves in addition to those emitted by the infrared source, thanks to the collisions between electrons, photons and atoms. When they come into phase, these waves form light pulses that are shorter than those offered by the laser, on the order of attoseconds.
According to the theory, as the laser penetrates the gas, it modifies the electromagnetic field holding the electrons to their atoms. Some of these electrons are then torn away from their atomic nuclei, without being completely ejected. These electrons are eventually recaptured by the nucleus. In so doing, they release energy in the form of a flash of light lasting a few attoseconds, emitted in the XUV spectrum (article 2).
This paved the way for new experiments to describe and qualify the phenomenon. For example, how could we demonstrate that these pulses are indeed so short, when there is no equally fast stopwatch?
In 2001, almost 15 years after Anne L'Huillier's observations, Pierre Agostini's experimental protocol made it possible to measure a train of flashes, and to record a duration of 250 attoseconds for each one (article 3). A world first. To achieve this result, the researcher ionized atoms with these flashes to create electronic replicas of them. He then used part of the original laser beam, synchronized with the XUV flashes, to manipulate these replicas in time. The properties of these electron packets, which are easier to measure, bear the imprint of the flashes and thus determine their duration. At the same time, Ferenc Krausz (third recipient of the 2023 Nobel Prize in Physics) and his group in Austria were working on a similar principle and succeeded in generating an isolated pulse lasting 650 attoseconds (article 4).
The development of a new attosecond method
Attosecond physics is now widely used to probe electronic processes. But the technique currently in use has several limitations, such as the low energy of the flashes produced. "If the initial light source is too powerful, there is a risk that the electrons in the gas will be completely freed from their orbits. This would make it impossible to obtain the coveted XUV flashes", explains Rodrigo Lopez-Martens. This is why LOA researchers prefer plasmas over noble gases. Highly ionized by nature, this state of matter frees them from the delicate equilibrium at play, enabling them to use much more intense lasers.
The team is thus developing a very powerful light source, unique in the world, and studying its effects at close quarters. "By directing our laser onto a solid surface, we form a plasma in which enormous forces appear, accelerating electrons to the speed of light. The plasma then acts like a mirror which, by also vibrating at the speed of light, adds new, shorter waves to the original laser light. It's a more efficient mechanism for generating attosecond flashes", adds Stefan Haessler. For the record, the first foundations of this technique in plasmas were laid in the late 70s. "It was a paper that went completely unnoticed at the time and no one followed up on it", smiles Rodrigo Lopez-Martens. Science sometimes has a few surprises in store.
Potential applications
Most of this work is carried out in the field of fundamental research. Nowadays, physicists know how to write the exact equations of quantum theory that govern the dynamics of a system with many electrons, but solving them remains a complex task. Computers struggle to handle any atom larger than helium. So the theory has to be simplified and approximated, then validated by comparison with experiments on these "too-big" atoms. Being able to observe in real time the movement of an electron (or several electrons) in interaction with all the counterparts in its atom, then provides invaluable data.
In 2002, for example, an article by Ferenc Krausz's team showed that attosecond physics had made it possible to track the emission of an Auger electron; an electron emitted when an atom previously bombarded by an ionizing ray is de-excited. Twenty years later, French scientists were able to reconstruct and "film" the dynamics of electron emission in the photoelectric effect (editor's note: in which the absorption of a photon by an atom is accompanied by the almost instantaneous emission of an electron).
Understanding the correlated dynamics of electrons is at the root of many microscopic processes and electrical currents involved in the decisive stages of chemical reactions. It goes far beyond fundamental atomic physics.
"In an organic protein such as rhodopsin (editor's note: a molecule found in the retina and involved in the eye's sensitivity to light), energy transport is conditioned by the movement of electrons between atoms and their role in the various atomic bonds. Observing electron packets using attosecond physics means understanding where the energy goes in the protein, and seeing if it's possible to direct it in a specific way to act on the function of this molecule", enthuses Rodrigo Lopez-Martens. We're aiming for applications in biology, medicine, pharmacology...
Fascinating prospects can also be imagined in condensed matter physics, where it is now possible to modify the electronic properties of a solid in the space of a very brief instant (less than a femtosecond, or 10-15s). "We imagine we could lay the foundations for a new generation of electronics operating at frequencies 100,000 times higher than the current state of the art (of the Petahertz order, i.e. 1015 Hz). Paradoxically, this horizon is still a long way off", concludes the researcher.
*LOA: a joint research unit CNRS, École Polytechnique, ENSTA Paris, Institut Polytechnique de Paris, 91120 Palaiseau, France
References