Creating and analysing superionic ammonia in the lab
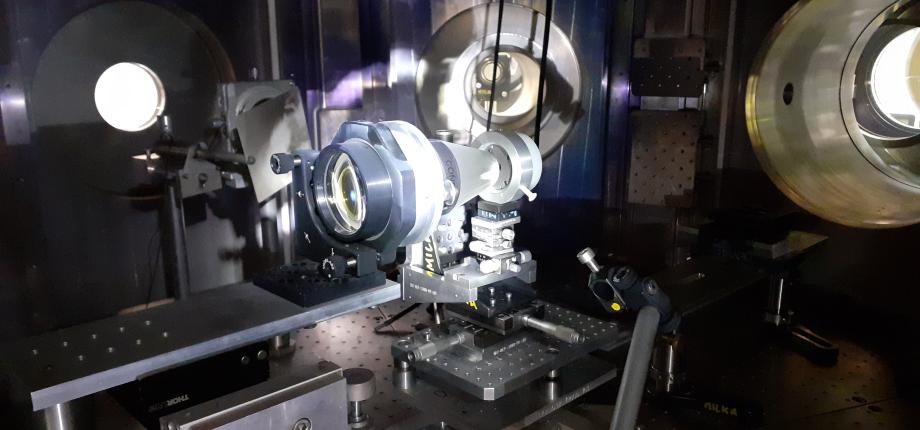
Uranus and Neptune are the least-known planets in the Solar System. Apart from a flyby by the Voyager 2 probe in the 1980s, no space mission has studied them closely. Scientists nevertheless believe that, beneath an atmosphere of hydrogen and helium, these ice giants have a mantle made up of water, methane and ammonia. Under the very high pressures and temperatures inside these planets, the water and ammonia could be in a state known as "superionic" ice. However, current models of Uranus and Neptune often consider water as the only component of their mantle, which does not seem sufficient to describe their structure and explain, for example, their unusual magnetic field, which is thought to be due to the movements of conducting fluids in the mantle. Experiments conducted at the Laboratoire pour l'Utilisation des Lasers Intenses (Intense Lasers Laboratory LULI*) by an international collaboration, combined with theoretical calculations, have succeeded in studying ammonia in these extreme conditions.
Static and dynamic compression
This superionic phase of ammonia had previously been observed at the Institut de Minéralogie, de Physique des Matériaux et de Cosmochimie (IMPMC), but its properties in planetary interior conditions remained unexplored until now. Reaching these pressures and temperatures (several million times higher than the Earth's atmospheric pressure and several thousand degrees) is indeed a challenge. Traditional methods, which involve either compressing the ammonia between two small 'anvils' made of a highly resistant material such as diamond or sapphire - static compression - or creating a shock wave in the sample using a laser - dynamic compression - are not enough on their own. The experiment therefore combines the two approaches: ammonia is first inserted and pre-compressed between two anvils at the IMPMC, until it is transformed into a liquid or even solid state. Then, the LULI2000's intense laser beam is sent through one of the anvils, creating a shock wave that heats and further compresses the sample. The data collected can be used to determine changes in temperature as a function of pressure, as well as other parameters such as the reflectivity of the ammonia, which can be used to determine its electrical conductivity. "These experiments, which combine LULI's expertise in dynamic compression and IMPMC's expertise in static compression, are conducted in close synergy with ab initio theoretical calculations carried out in collaboration with the geology laboratory in Lyon and the University of Rostock," explains Alessandra Ravasio, a CNRS researcher at LULI. These theoretical calculations involve using the first principles of physics to determine how the ammonia sample behaves under these conditions. The first experiments test the validity of the calculations, which then help to interpret the subsequent experiments.
The results of these new experiments were published in a Nature Physics article, with Jean-Alexis Hernandez (a post-doctoral fellow at LULI at the time of the experiment) as first author. They make it possible to explore new states of ammonia, in particular its "superionic ice" phase. In this phase, ammonia (a molecule made up of one nitrogen atom and three hydrogen atoms) has properties common to both a solid and a liquid. The nitrogen atoms form an organised network (as in a solid), while the hydrogen atoms move rapidly in a more chaotic fashion (as in a liquid). For certain initial conditions associated with pre-compressions of a few gigapascals (1 gigapascal is equivalent to 10,000 atmospheric pressures), the researchers observed a rather unusual pattern of temperature versus pressure: the temperature increases very little, while the pressure continues to rise. Above a certain pressure, the rate of temperature change increases again. These "slope breaks" are typical of a phase transformation in which the energy supplied to the ammonia does not cause the temperature to rise, but fuels the change of state. "In our case, this would be the transition from the superionic phase to the plasma fluid phase present at a higher temperature," explains Alessandra Ravasio. This interpretation of the results as showing the fusion of superionic ice was validated by theoretical calculations.
Melting of superionic ammonia
These results, combined with the team's previous work, make it possible to plot the melting curve of superionic ammonia over a wide range of pressures, and show that above 100 gigapascals, superionic ammonia melts at a lower temperature than superionic water ice. "This could lead to the melting of ammonia-rich regions in the mantles of Uranus and Neptune, compared with models that only take water into account," says Alessandra Ravasio. In addition, the results show that ammonia has a higher electrical conductivity than water under the conditions of the Uranus and Neptune interior models.
All these results provide crucial information for revising the internal structure of the ice giants and for estimating the extent of the region in which their unusual magnetic field can be generated. Scientists hope to continue in this direction, for example by studying a mixture of ammonia and water, or even adding methane to get as close as possible to real conditions. New challenges lie ahead.